This post is a continuation of our in-depth look at the subject of nuclear fusion power.
Here we will look at the issues and difficulties of creating a physical system in which we can create the conditions necessary for nuclear fusion. This post can be regarded as an introduction to the realities of fusion reactor design.
To successfully construct a nuclear fusion reactor, we must face temperatures that vaporize all known materials. We must also engineer a way around other problems that make this the most difficult technological endeavor of our age. This piece is an starting point only, and does not claim to delve into the full breadth and depth of fusion implementation issues.
Before we start, some readers may find it very helpful to read about:
- An introduction to how humans hope to harness fusion power.
- Helpful tools for understanding nuclear fusion power.
- Fusion fuel cycles: What are they and how do they work?
People with an inclination towards science learning may also be interested in the science behind fusion power.
Contents
Starting points
We are attempting to address the problem of creating fusion conditions through the use of machinery on earth. Well, what do we know about already functioning fusion reactors?
Our sun is an excellent example of fusion energy in action. The problem is, the core of the sun, where most of its fusion reactions are taking place, is at around fifteen million degrees kelvin1 , and is under an estimated pressure of at least 3.4 x 108 atmospheres2 . Not a very hospitable place.
With current methods we are simply unable to maintain pressures anywhere near that high with our machinery on earth. We can do it for short periods of time in nuclear weapons, but essentially nowhere else.
Luckily, we are capable of creating much higher temperatures than those found in the center of the sun. What we lack in pressure we may be able to make up for with heat. Fusion systems today are looking at creating temperatures well into the hundreds of millions of degrees kelvin. For instance, the Deuterium-Tritium fusion fuel cycle hits its maximum fusion rate at around 800 million degrees kelvin3 , and hydrogen-boron fusion hits its max at around 6.6 billion kelvin4 .
So we begin our discussion with how we can possibly conceive of containing plasma that is at such incredible temperatures. Luckily nature is kind, and there are a few ways.
Electromagnetism to the rescue
With fusion we are talking about temperatures so high that all materials are gaseous plasma. There is no way to build a physical vessel that can contain these temperatures. Luckily there is another way, by using electromagnetism.
The electric force is easier to understand than magnetic. When people think of ‘magnets’ they think of things sticking to each other or repelling each other. These are actually the properties of the electric force, not of the magnetic force.
If you want to dig into the subject, you can read about how the electric force is a straight push or pull on an electric charge. Like charges repel, and opposite charges attract.
The magnetic force however is much less obvious. A constant magnetic field causes moving charges to change the direction of their motion. This is why a magnetic field can cause charged particles to move in a circle or spiral.
Looking at these forces together is educational. Wikipedia has a nice article on the Lorentz force, which is the total electromagnetic force on a charged particle.
Magnetic pressure
Magnetic pressure refers to the fact that we can use magnetic fields in specific geometries to create a contained space. Magnetic pressure refers to the strength of the confinement that we are creating.
Those people who have studied electromagnetism may know that charged particles tend to spiral around magnetic field lines.5 Thanks to this effect, magnetic fields in certain configurations can exert a pressure on ionized plasma to keep it contained.
We will look at the tokamak in detail in a later post. It is a fusion reactor design that uses a very specific geometrical shape (similar to a donut) that is useful for containing plasmas.
Electrostatic confinement
Fuel ions are positively charged. That is, they are lacking one or more of their usual complement of electrons. How do we contain something that is positively charged?
We create a strong negative charge near the center of the reactor. The ions will be strongly attracted to it, and will form a rough ball of plasma ions.
This is a simplistic picture, since obviously the ions are going to hit the charge at the center and steal some electrons, then becoming neutral gas. In a later post we will be looking at the Farnsworth-Hirsch Fusor and the Polywell as illustrative examples of how we can achieve fusion using this technique.
Power density
Power density is the power divided by the volume. Using a number of simplifying approximations, we can write power density as:
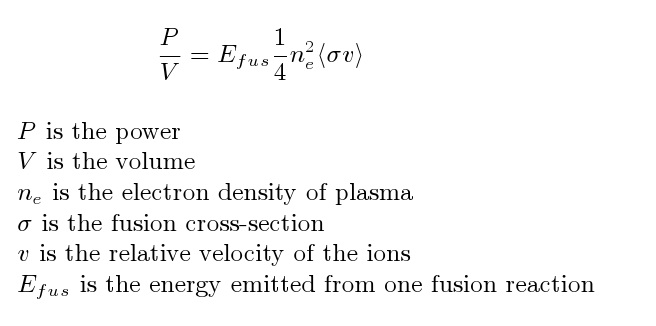
We can also say that power density is defined as the power produced per unit volume. This equation operates under a number of assumptions. In this case it assumes that there are two different types of reactant, and that they are present in equal densities. Also we are assuming that the electron density is equal to the total ion density. The angle brackets mean an average over the Maxwellian velocity distribution.6
Power density can be a useful metric for ascertaining the feasibility of a fusion power system. This is because a low power density means that we would have to build a very large system to produce significant amounts of energy. Lower power density can be roughly correlated with higher cost. It should be kept in mind of course that this is just one of many factors that contributes to the feasibility or cost of a proposed fusion power plant. Just as with other forms of electrical power generation, there are a myriad of things to consider such as materials, longevity, environmental effects, health effects, capacity factor, dispatchability, and safety.
Reactor construction materials
The materials considered for use in most fusion reactor designs need to be able to withstand very high neutron flux. This is primarily due to the fact that D-T is the most popular fuel cycle, and it produces 80% of its energy in the form of high-energy neutrons. Materials used near the core are going to get hammered by neutrons emitted from the fusion core.
High neutron flux can create some engineering difficulties in terms of activated materials and reaction products. Activated materials are isotopes that were not originally radioactive, but have become so after being bombarded with neutron radiation.
Humans have a fair bit of experience in this area thanks to fission reactors. I have found various references over the months to the amount of neutron radiation from fusion power. In a review article some time ago, R. F. Post said that in a fusion reactor, the neutron flux may be 1000 times as high as in a fission-based Pressurized Water Reactor(PWR). The Wikipedia article for fusion power currently states that it would be about 100 times as high as a PWR. I haven’t found any newer citations to this effect. At the very least, we can expect to have neutron fluxes at least on the same order of magnitude as fission reactors.
Divertor systems are a method for trying to take the fusion products out of the plasma in tokamaks. The energy flux coming through a divertor may be tremendous. Some sources have listed it as high as 100 MW per square meter.
There is no known material that can handle this amount of energy flux without being destroyed. The hope is to create diverging magnetic field lines to disperse the particles, and their energy, over a much wider surface area. This is expected to be possible, though perhaps difficult to engineer in practice. This is one of several technical hurdles that the tokamak design will have to surmount in the next few decades.
Fusion power to electricity
There are two major techniques for using fusion power to electricity. Each of these converts fusion energy to electricity using a different method and with some efficiency.
The first of these is a well-known technique using heat engines such as steam turbines which can reach an efficiency of 33-50% with current technologies.
The other is a relatively new technique that utilizes a very different perspective. It is called ‘direct conversion‘, and it may present a way to reach efficiencies above 80%.
We have completed an in-depth article on these two methods for converting fusion energy to electricity.
- “The Sun’s Interior: Core”. HowStuffWorks. Accessed November 10th, 2010. [↩]
- Pressure at the Center of the Sun. The Physics Factbook. Edited by Glenn Elert. Accessed November 10th, 2010. [↩]
- Wikipedia: Nuclear Fusion. Accessed November 10th, 2010. [↩]
- Wikipedia: Aneutronic fusion. Accessed November 10th, 2010. [↩]
- Basic facts about spiral motion. Windows to the universe. [↩]
- Wikipedia: Maxwell–Boltzmann distribution. Accessed October 20th, 2010. [↩]
4 thoughts to “The basic realities of fusion reactor design”